Lecture 20Introduction to photosynthesis
|
Introduction to photosynthesis
General
Carbon cycle and Global Change
Light Harvesting
Overview of light harvesting
At ambient intensities, an antenna consisting of 1 chlorophyll molecule would intercept a photon once every few seconds. The decay rates of some of the energy storage mechanisms (charge separation in the photochemical reactions, the proton gradient) have half-times in this range, so photosynthesis with an antenna this size would be inefficient. In order to achieve an efficient use of light energy, the photosynthetic organisms have evolved light harvesting antenna, which allow many pigments to cooperate in the collection of light for a single reaction center. In many photosynthetic systems, the size of the antenna can be adjusted to suit the intensity of light. An effective antenna is particularly important for growth at low light intensity.
Click here for a brief review of antenna function by Bob Blankenship.
Transfer of excitation from the antenna to the reaction center occurs with high efficiency. Evolution has designed structures which meet the requirements of physics and chemistry, as determined by the absorption in the atmosphere, the potential for photochemical damage, and the requirements for efficient transfer as described by Forster theory for energy exchange between neighboring pigments (see below). The requirements are:
- Molecules must absorb in a region of the spectrum where light reaches the surface of the planet with sufficient intensity. Since CO2 and water vapor absorb much of the far IR, and oxygen and ozone absorb all the far UV, this limits the range to the visible and near IR.
- The light must have sufficient energy for useful chemistry, but not be destructive (the former limits the range to < 1000 nm (the near IR), the latter to >340 nm (the near UV).
- For energy transfer, the molecules must:
- be the right distance apart
- be appropriately aligned
- have overlapping spectra for fluorescence and absorption
- For efficient energy transfer, the system is usually organized with a thermodynamic gradient from antenna to reaction center (an energy "funnel") . This means that the peripheral antenna absorbs light of higher energy (to the blue end of the spectrum), and transfers excitation to pigments with lower energy absorbance bands (further to the red), with the photochemical trap (the reaction center) at the lowest energy (furthest to the red). Although most photosynthetic systems follow this pattern, in some cases, evolution has adapted this plan to take advantage of other considerations. Most important is the fact that photosynthetic systems compete for light, so an organism is at an advantage if it can find a niche in the spectrum underused by its competitors.
Fluorescence and light harvesting
Fluorescence from photosynthetic systems varies with the physiological state, and measurement of fluorescence is much used as a tool for exploring mechanism. General principles are covered here, and use of fluorescence to explore mechanism in photosynthesis in Lecture 21.
The Forster mechanism of excitation transfer
A brief description of the main parameters of Forster mechanism for inductive resonance transfer is given here.
Bacterial light harvesting
The structure of the light harvesting complex LH2 from a photosynthetic bacterium has recently been solved by X-ray crystallography by RRichard Cogdell and colleagues. Click here for a nice introduction to the structure of the bacterial light harvesting complex from Rhodopseudomonas acidophila, pictures from the Nature article, and a Chime tutorial on the structure from Richard Cogdell's group.
Click here for a review of bacterial light harvesting complexes from the Schulten lab, which gives a nice overview of their recent structural and theoretical work.
Click here for an overview of work on the light harvesting complex from Rs. molischianum, from Schulten's group.
The Rhodopseudomonas acidophila complex is typical of the light harvesting complexes from the purple bacteria, and contains two small protein subunits, a and b, three bacteriochlorophylls (BChl), and two carotenoids. The spectrum of the complex shows two BChl peaks in the near IR, at 800nm and at 850 nm. These correspond to 1 B800 BChl, and two B850 BChls.
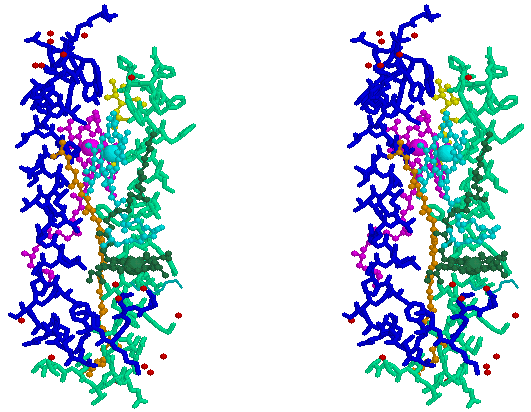
Stereo-pair view of a single a, b-subunit pair from the light harvesting complex from Rhodopseudomonas acidophila. The BChl of B800 is shown as dark green, the B850 pair is shown as cyan and purple, carotenoids are orange and yellow, water oxygens are red; a-subunit is blue, b-subunit is pale green. For a Chime tutorial on the structure, click here. Coordinates kindly provided by Dr. Cogdell.
In the membrane (and also in the crystals), the complete protein is made of 9 such a, b-subunit pairs, arrange in a cylinder, as seen in the links above, and this image from the Cogdell lab.
and this one, which has 8 a, b-subunit pairs, from Schulten's lab:
These cylindrical assemblies allow the B850 BChls to form a closely coupled ring in which excitation transfer by an exciton mechanism is very rapid. Excitation transfer in the B800 BChl ring, and between B800 and B850 molecules, is less rapid, but still on the fast end of Forster theory.
A structure for the LH1 complex from Rhodospirillum rubrum at ~10 Å resolution has been solved by electron diffraction crystallography by Robin Ghosh and colleagues. On the basis of this structure and the sequence similarity, it is clear that the LH1 forms a similar structure with a, b-subunit pairs as in LH2, but with only the two BChls (called B875) corresponding to B850. Ghosh has pointed out that the LH1 supercomplex has 16 a, b-subunit pairs in its cylinder. This is sufficient to contain 1 reaction center within the pallisade. Cogdell and colleagues, in collaboration with Werner Kühlbrandt, have recently solved a low resolution electron diffraction structure for the LH1-reaction center complex, which confirms Ghosh's suggestion.
In the bacterium, the LH1 complexes are synthesized in a fixed, and the LH2 complexes in a variable stoichiometry, with respect to the reaction center. The LH2 proteins are coded by the puc-operon, and the LH1 subunits, and the M and L subunits of the reaction center, are coded by the puf-operon. The two operons are under different control, so that the LH2 complex is expressed in greater amounts at lower light intensities.
In the complete photosynthetic apparatus, the LH2 structures lie outside the LH1 pallisade, but the B850 ring at the same level in the membrane as the B870, which line up with the ancillary BChls of the reaction center, to provide a pathway for rapid excitation transfer from the peripheral antenna to the BChl-dimer (P865) of the reaction center. This picture, adapted from an image from Richard Cogdell's lab, shows a cross section through a model structure containing all three complexes.
A similar model based on the Rs. molischianum structure, from Schulten's group:
Light harvesting in green plants
The light harvesting antenna of photosystem II consists of three main groups of proteins.
- The photosystem II reaction center as normally isolated contains two main subunits, CP43 and CP47, which bind chlorophyll a as a "core" antenna.
- The bulk antenna consists of trimers of LHCIIb, the structure of which is solved from electron diffraction crystallography (see below).
- The bulk antenna is connected to the core antenna through the minor LHC complexes. These are LHCIIa, c and d (the letters a,b,c and d refer to the order of apparent molecular weights on gels), also called CP29, 26, and 24 (CP - chlorophyll protein, where the number indicates the Mr on gels).
The structure of light harvesting complex II (LHCII)
This is a brief tutorial on the structure of LHCII.
The tutorial uses Chime, which you can download from here.
The role of the minor light harvesting complexes in photoprotection
In addition to their antenna function, the minor light harvesting complexes have an additional role in protection of the photosynthetic apparatus against bright light. Plants have evolved to operate most efficiently at light intensities corresponding to scattered sunlight. In full sunlight, the intensity is several-fold brighter than that necessary to saturate photosynthesis. In the absence of protective mechanisms, the photosystems get destroyed by photo-oxidative damage. Protection occurs through a switch, which changes the function of the minor CPs from excitation transfer to exciton dumping; the energy is lost as heat rather than passed to the reaction center. The switching depends on two main factors:
- The xanthophyll carotenoid, violaxanthin, is converted to antheraxanthin and zeaxanthin by de-epoxidation. The de-epoxidase is turned on by a fall in the lumenal pH.
- In complexes which contain the de-epoxidation products, lowering of the lumenal pH causes the configuration of pigments to change so as to introduce the exciton dumping pathway.
The drop in lumenal pH is the feed-back signal from metabolism; as the light intensity delivers reducing power and ATP faster than the CO2-fixation reactions can handle it, the ATP synthase backs-up, causing the DpH to build up, leading to the drop in lumenal pH which triggers the protective mechanism.
For a more extensive discussion, click here.
Kinetics of light harvesting to photosystem I
On-line lecture on The First 100 Picoseconds of Photosynthesis from Tom Owens' lab.
©Copyright 1996,
Antony Crofts, University of Illinois at Urbana-Champaign,
a-crofts@uiuc.edu